Nuclear Energy
Introduction
"The discovery of nuclear chain reactions need not bring
about the destruction of mankind any more than did the discovery of
matches. We only must do everything in our power to safeguard against
its abuse."
Albert Einstein
One of the most mysterious phenomena in the universe is the
conversion of mass into energy. The whole universe is "powered" by
this process. The energy radiated by stars, including the Sun,
arises from nuclear reactions (called fusion) deep in their interiors.
The release of nuclear energy occurs through the fusion of two light
hydrogen nuclei into a heavier nucleus of helium.
SOHO: The Solar and Heliospheric Observatory
Until about 1800, the principal fuel on our planet was wood, its
energy originating from solar energy stored in plants during their
lifetimes.
Since the Industrial Revolution, people have depended on fossil fuels—coal,
petroleum, and natural gas—also derived from stored solar energy. When a
fossil fuel such as coal is burned, atoms of hydrogen and carbon in the
coal combine with oxygen atoms in air. Water and carbon dioxide are
produced and heat is released. This amount of energy is typical of
chemical reactions resulting from changes in the electronic structure of
the atoms. A part of the energy released as heat keeps the adjacent fuel
hot enough to keep the reaction going.
In a nuclear reaction, however, the energy released is often about 10
million times greater than in a chemical reaction, and the change in
mass can easily be measured.
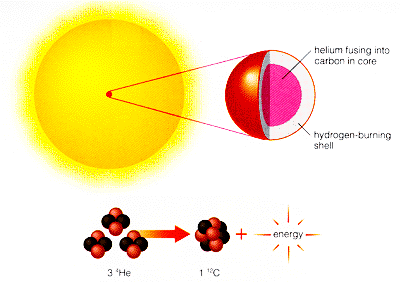
History
The history of generating huge amounts of energy in a nuclear
reaction, is basically the history of the atom bomb...Here are few
important steps that led to the release of nuclear energy on demand.
- In 1896, Antoine Henri Becquerel discovered radioactivity
in uranium.
- In 1902, Marie and Pierre Curie isolated a radioactive metal
called radium
- In 1905, Albert Einstein formulated his Special Theory of
Relativity. According to this theory, mass can be
considered to be another form of energy. According to Einstein, if
somehow we could transform mass into energy, it would be possible to "liberate"
huge amounts of energy.
- During the next decade, a major step was taken in that direction
when Ernest Rutherford and Niels Bohr described the structure of an
atom more precisely. It was made up, they said, of a
positively charged core, the nucleus, and of negatively charged
electrons that revolved around the nucleus. It was the nucleus,
scientists concluded, that had to be broken or "exploded" if atomic
energy was to be released.
- In 1934, Enrico Fermi of Italy disintegrated heavy atoms by
spraying them with neutrons. However he didn't realize that he had
achieved nuclear fission.
- In December 1938, though, Otto Hahn and Fritz Strassman in Berlin
did a similar experiment with uranium and were able to verify a world-shaking
achievement. They had produced nuclear fission (they had split
an atom)
- 33 years after Einstein said it could be done mass was transformed
into energy.
- On August 2, 1939, Albert Einstein wrote a letter to the
American President, Franklin D. Roosevelt. "In the course of
the last four months, it has been made probable - through the work of
Joliot in France as well as Fermi and Szilard in America - that it may
become possible to set up nuclear chain reactions in a large mass of
uranium... And this new phenomenon would also lead to the construction
of bombs... A single bomb of this type, carried by boat or exploded in
a port, might very well destroy the whole port together with some of
the surrounding territory." He urged Roosevelt to begin a
nuclear program without delay.
In later years Einstein deplored the role he had played in the
development of such a destructive weapon: "I made one great mistake
in my life," he told Linus Pauling, another prominent scientist,
"when I signed the letter to President Roosevelt recommending that
atoms bombs be made."
- In December 1942 at the University of Chicago, the Italian
physicist Enrico Fermi succeeded in producing the first nuclear
chain reaction. This was done with an arrangement of natural
uranium lumps distributed within a large stack of pure graphite, a
form of carbon. In Fermi's “pile,” or nuclear reactor, the graphite
moderator served to slow the neutrons.
- In August 1942, during World War II, the United States established
the Manhattan Project. The purpose of this project was
to developed, construct, and test the A-bomb. Many prominent American
scientists, including the physicists Enrico Fermi and J. Robert
Oppenheimer and the chemist Harold Urey, were associated with the
project, which was headed by a U.S. Army engineer, then-Brigadier
General Leslie R. Groves.
- On May 31, 1945, sixteen men met in the office of Secretary of War
Henry L. Stimson. The sixteen men were there to make decisions
about a weapon the average American had never heard of - the
atom bomb. They picked future targets for "The Bomb." They were
not talking about "just another weapon." What they were discussing was
"a new relationship of man to the universe," as said by Stimson.
Humankind, the Secretary seemed to be saying, was at the most critical
turning point in its entire recorded history.
The super-secret group also had many questions about the future
including:
- What were the chances of producing nuclear weapons more powerful
that the one being developed?
- How long would it take other nations, especially the Soviet
Union, to catch up with the United States?
- What hope was there to use atomic energy for peaceful purposes,
such generating as electricity?
- On July 16, 1945, the first atomic bomb (or A-bomb), was
tested at Alamogordo, New Mexico.
- On August 6, 1945, the Enola Gay, an American
airplane, dropped the first atomic bomb ever used in warfare on
Hiroshima, Japan, eventually killing over 140,000 people. On
August 9, 1945, the United States dropped a second atomic bomb, this
time on the Japanese city of Nagasaki. The drop is one mile off target,
but it kills 75,000 people.
- On August 29, 1949, the Soviet Union tested its first atomic
device at the Semipalatinsk test range. (Up to 20kt yield)
- On November 1, 1952, a full-scale, successful experiment
was conducted by the United States with a fusion-type device.
- In 1946, the Atomic Energy Commission (AEC), civilian agency of
the United States government, was established by the Atomic Energy Act
to administer and regulate the production and use of atomic power.
Among the major programs of the new commission were production of
fissionable materials; accident prevention; research in biology,
health, and metallurgy and production of electric power from the atom;
studies in the production of nuclear aircraft; and the
declassification of data on atomic energy.
The most important goal of the 1946 act, however, was to put the
immense power and possibilities of atomic energy under civilian
control, although nuclear materials and facilities remained in
government hands.
- A revised Atomic Energy Act in 1954 allowed for licensed private
ownership of facilities to produce fissionable materials.
- In 1964 an amendment permitted private ownership of nuclear fuels,
which aided the growing nuclear power industry.
- Under the Energy Reorganization Act of October 1974, the AEC was
abolished, and two new federal agencies were established to administer
and regulate atomic-energy activities: the Energy Research and
Development Administration and the Nuclear Regulatory Commission.
- In 1977, the responsibilities of the former were transferred to
the newly established Department of Energy.
The Equivalence of Mass and Energy
In 1905, Albert Einstein formulated his Special Theory of
Relativity. According to this theory, mass can be considered
to be another form of energy.
In our daily life, mass and energy seem to be separate. In a
nuclear reaction, however, the energy released is often about a million
times greater than in a chemical reaction, and the change in mass
can easily be measured.
Mass and energy are related by what is certainly the best-known equation
in physics:
E=mc2
in which E is the energy equivalent (called mass energy) of mass m,
and c is the speed of light.
A very small amount of matter is equivalent to a vast amount of
energy.
For example, 1 kg (2.2 lb) of matter converted
completely into energy would be equivalent to the energy released by
exploding 22 megatons of TNT.
--------------------------------------------------------------------------------
"And Jesus said unto them, Because of your unbelief: for
verily I say unto you, If ye have faith as a grain of mustard seed, ye
shall say unto this mountain, Remove hence to yonder place; and it shall
remove; and nothing shall be impossible unto you."
Jesus
Matthew, 17:20, The King James Bible
Nuclear Energy
Nuclear Energy is released during the splitting (fission) or fusing
of atomic nuclei. The energy of any system, whether physical, chemical,
or nuclear, is manifested by the system's ability to do work or to
release heat or radiation. The total energy in a system is always
conserved, but it can be transferred to another system or changed in
form. According to the Law of Conservation of Energy, if
we add up the total amount of energy in the universe (we can describe
energy quantitatively with units such as Joules or kilowatt-hours), the
total amount never changes. In other words, energy is neither created
nor lost, even though it may be converted from one form to another.
Thus the law of conservation of energy is really the law of conservation
of mass-energy.
The Atom
The atom consists of a small, massive, positively charged core,
nucleus, surrounded by electrons.
The nucleus contains most of the mass of the atom. It is itself
composed of neutrons and protons.
- The proton is 1,836 times as heavy as the electron.
For an atom of hydrogen, which contains one electron and one proton,
the proton provides 99.95 percent of the mass.
- The neutron weighs a little more than the proton.
Elements heavier than hydrogen usually contain about the same number
of protons and neutrons in their nuclei, so the atomic mass, or the
mass of one atom, is usually about twice the atomic number.
Protons are affected by all four of the fundamental forces
that govern all interactions between particles and energy in the
universe.
- The electromagnetic force arises from matter carrying an
electrical charge. It causes positively charged protons to attract
negatively charged electrons and holds them in orbit around the
nucleus of the atom.
- The strong nuclear force binds the protons and neutrons
together into a compact nucleus. This force is 100 million times
stronger than the electrical attraction that binds the electrons. It
must be strong enough to overcome the repulsive force of the
positively charged nuclear protons and to bind both protons and
neutrons into the tiny nuclear volume. The nuclear force must also be
of short range because its influence does not extend very far beyond
the nuclear "surface." The nuclear force is due to a strong force that
binds quarks together to form neutrons and protons.
- The other two fundamental forces, gravitation and the weak nuclear
force, also affect the proton. Gravitation is a force that
attracts anything with mass (such as the proton) to every other thing
in the universe that has mass. It is weak when the masses are small,
but can become very large when the masses are great.
- The weak nuclear force is a feeble force that occurs
between certain types of elementary particles, including the proton,
and governs how some elementary particles break up into other
particles.
Chemical Elements
The number of protons in the nucleus of an atom determines what kind
of chemical element it is. All substances in nature are made up
of combinations of the 92 different chemical elements, substances that
cannot be broken into simpler substances by chemical processes. The atom
is the smallest part of a chemical element that still retains the
properties of the element. The number of protons in each atom can range
from one in the hydrogen atom to 92 in the uranium atom, the heaviest
naturally occurring element.
For a very different and controversial theory
of the atom
visit The Living Atom
Theory
Another controversial theory:
Metaparticles
The Nucleus
The nucleus contains most of the mass of the atom. It is
itself composed of neutrons and protons bound together by very
strong nuclear forces, much greater than the electrical forces that
bind the electrons to the nucleus. The nucleus of an atom is described
by these characteristics:
- The mass number "A" of a nucleus is the number of nucleons
(protons and neutrons) it contains.
- The atomic number "Z" is the number of positively charged
protons.
For example, the expression
U, represents
uranium with A=235 (number of nucleons) and Z=92 (number of protons).
- The binding energy of a nucleus is a measure of how tightly
its protons and neutrons are held together by the nuclear forces.
The binding energy per nucleon, the energy required to remove
one neutron or proton from a nucleus, is a function of the mass
number A.
The total energy required to break up a nucleus into its
constituent protons and neutrons can be calculated from
,
called nuclear binding energy.
If we divide the binding energy of a nucleus by the number of protons
and neutrons (number of nucleons), we get the binding energy per nucleon.
This is the common term used to describe nuclear reactions because
atomic numbers vary and total binding energy would be a relative term
dependent upon that. The following figure, called the binding energy
curve, shows a plot of nuclear binding energy as a function of mass
number. The peak is at iron (Fe) with mass number equal to 56.
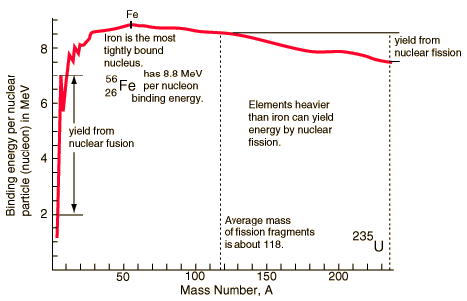
The binding energy per nucleon is a
function of the mass number
The curve of binding energy implies that if two light
nuclei near the left end
of the curve coalesce to form a heavier nucleus (the process is called
fusion),
or if a heavy nucleus at the far right splits into two lighter ones (the
process is
called fission), more tightly bound nuclei result, and energy
will be released.
The rising of the binding energy curve at low mass numbers, tells us
that energy will be released if two nuclides of small mass number
combine to form a single middle-mass nuclide. This process is called
nuclear fusion.
The eventual dropping of the binding energy curve at high mass
numbers tells us on the other hand, that nucleons are more tightly bound
when they are assembled into two middle-mass nuclides rather than
into a single high-mass nuclide. In other words, energy can be released
by the
nuclear fission, or splitting, of a single massive nucleus into two
smaller fragments.
The binding energy curve shows that energy can be released if two
light nuclei combine to form a single larger nucleus. This process is
called nuclear fusion. The process is hindered by the electrical
repulsion that acts to prevent the two particles from getting close
enough to each other to be within range and "fusing."
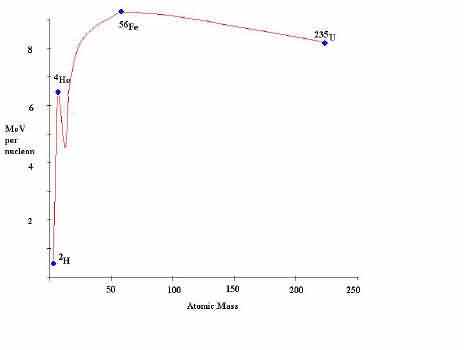
To generate useful amounts of power, nuclear fusion must occur in bulk
matter. That is, many atoms need to fuse in order create a significant
amount of energy. The best hope for bringing this about is to raise the
temperature of the material so that the particles have enough energy -
due to their thermal motions alone - to penetrate the electrical
repulsion barrier. This process is known as thermonuclear fusion.
Calculations show that these temperatures need to be close to the
sun's temperature of 1.5 X 107K.
Nuclear energy, measured in millions of electron volts (MeV),
is released by the fusion of two light nuclei, as when two heavy
hydrogen nuclei, deuterons, combine in the reaction
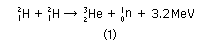
producing a helium-3 atom, a free neutron, and 3.2 MeV (3.2x106eV).
Although the energy release in the fusion process is
less per nuclear reaction than in fission, 0.5 kg (1.1 lb) of the
lighter material contains many more atoms; thus, the energy liberated
from 0.5 kg (1.1 lb) of hydrogen-isotope fuel is equivalent to that of
about 29 kilotons of TNT, or almost three times as much as from
uranium. This estimate, however, is based on complete fusion of all
hydrogen atoms. Fusion reactions occur only at temperatures of several
millions of degrees, the rate increasing enormously with increasing
temperature; such reactions consequently are known as thermonuclear (heat-induced)
reactions. Strictly speaking, the term thermonuclear implies that
the nuclei have a range (or distribution) of energies characteristic of
the temperature. This plays an important role in making rapid fusion
reactions possible by an increase in temperature.
Development of the hydrogen bomb was impossible before the perfection of
A-bombs, for only the latter could yield that tremendous heat necessary
to achieve fusion of hydrogen atoms. Atomic scientists regarded the A-bomb
as the trigger of the projected thermonuclear device.
Thermonuclear Fusion in the Sun and other Stars
The sun radiates energy at the rate of 3.9 X 1026 W (watts) and
has been doing so for several billion years. The sun burns hydrogen in a
"nuclear furnace." The fusion reaction in the sun is a multi-step
process in which hydrogen is burned into helium, hydrogen being the "fuel"
and helium the "ashes." Hydrogen burning has been going on in the sun
for about 5 billion years and calculations show that there is enough
hydrogen left to keep the sun going for about the same length of time
into the future. The burning of hydrogen in the sun's core is alchemy on
a grand scale in the sense that one element is turned into another.
Fusion takes place when the nuclei of hydrogen atoms with one proton
each fuse together to form helium atoms with two protons. A standard
hydrogen atom has one proton in its nucleus. There are two isotopes of
hydrogen which also contain one proton, but contain neutrons as well.
Deuterium contains one neutron while Tritium contains two. Deep within
the star, A deuterium atom combines with a tritium atom. This forms a
helium atom and an extra neutron. In the process, an incredible amount
of energy is released.
When the star's supply of hydrogen is used up, it begins to convert
helium into oxygen and carbon. As a star evolves and becomes still
hotter, other elements can be formed by other fusion reactions. If the
star is massive enough, it will continue until it converts carbon and
oxygen into neon, sodium, magnesium, sulfur and silicon. Eventually,
these elements are transformed into calcium, iron, nickel, chromium,
copper and others until iron is formed. When the core becomes primarily
iron, the star's nuclear reaction can no longer continue. Eements more
massive than those with atomic number equal to 56 (iron) cannot be
manufactured by further fusion processes as atomic number equal to 56
makes the peak of the binding energy curve. If nuclides were to fuse
after that, then energy would be consumed as opposed to produced.
The inward pressure of gravity becomes stronger than the outward
pressure of the nuclear reaction. The star collapses in on itself. What
happens next depends on the star's mass.
Nuclear energy is also released when the fission of a heavy
nucleus such as U is
induced by the absorption of a neutron as in
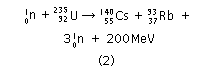
producing cesium-140, rubidium-93, three neutrons, and 200 MeV.
A nuclear fission reaction releases 10 million times as much energy
as is released in a typical chemical reaction. In practical units,
the fission of 1 kg (2.2 lb) of uranium-235 releases 18.7 million
kilowatt-hours as heat.
The fission process initiated by the absorption of one neutron in
uranium-235 releases about 2.5 neutrons, on the average, from the split
nuclei. The neutrons released in this manner quickly cause the fission
of two more atoms, thereby releasing four or more additional neutrons
and initiating a self-sustaining series of nuclear fissions, or a
chain reaction, which results in continuous release of nuclear
energy.
Using the
binding energy curve, we can estimate the energy released in this
fission process. From this curve, we see that for heavy nuclides (mass
about 240u), the mean biding energy per nucleon is about 7.6MeV. For
middle-mass nuclides (mass about 120), it is about 8.5 MeV. This
difference in total binding energy between a single large nucleus and
two fragments (assumed to be equal) into which it may be split is then
close to 200MeV. This is a relatively large amount of released energy
per fission event. When a chain reaction occurs, many atoms and nuclei
are involved so lots of energy is released.
Fission in Nuclear Reactors
To make large-scale use of the energy released in fission, one
fission event must trigger another, so that the process spreads
throughout the nuclear fuel as in a set of dominos. The fact that more
neutrons are produced in fission than are consumed raises the
possibility of a chain reaction. Such a reaction can be either rapid (as
in an atomic bomb) or controlled (as in a reactor).
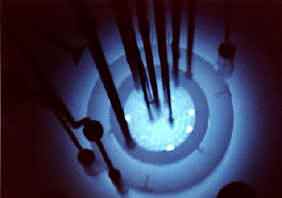
Core of the US Geological Survey (USGS)
nuclear research reactor, Triga II, while operating.
In a nuclear reactor, control rods made of cadmium or graphite or
some other neutron-absorbing material are used to regulate the number of
neutrons. The more exposed control rods, the less neutrons and vice
versa. This also controls the multiplication factor k which is the ratio
of the number of neutrons present at the beginning of a particular
generation to the number present at the beginning of the next generation.
For k=1, the operation of the reactor is said to be exactly critical,
which is what we wish it to be for steady-power operation. Reactors are
designed so that they are inherently supercritical (k>1); the
multiplication factor is then adjusted to the critical operation by
inserting the control rods.
An unavoidable feature of reactor operation is the accumulation of
radioactive wastes, including both fission products and heavy "transuranic"
nuclides such as plutonium and americium.
Nuclear Weapons
In the 20th century, the rapid advance of industry and modern
technology greatly increased both the destructive power of armed forces
and the capacity of societies both to resist and to recover from an
attack. Nuclear weapons carry the possibilities of destruction to a new
level and are able to inflict far greater damage within a few hours than
previously resulted from years of warfare. This not only makes the
consequences of war worse but also raises new concerns about controlling
such a destructive process. Indeed, nuclear weapons have not been
used in war since the first two atomic bombs were dropped on Japan in
1945, but many countries, including many Third World countries, now
have nuclear weapons.
Nuclear Weapons are explosive devices designed to release
nuclear energy on a large scale for military reasons. The first
atomic bomb (or A-bomb), was tested on July 16, 1945, at Alamogordo, New
Mexico.
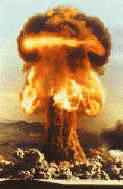 |
All explosives prior to that time derived their
power from the rapid burning or decomposition of some chemical
compound. Such chemical processes release only the energy of the
outermost electrons in the atom.
Nuclear explosives, on the other hand, involve energy sources within
the nucleus of the atom.
The A-bomb gained its power from the splitting (fission) of all the
atomic nuclei in several kilograms of plutonium. A sphere about the
size of a baseball produced an explosion equal to 20,000 tons of TNT. |
Although nuclear bombs were originally
developed as strategic weapons to be carried by large bombers, nuclear
weapons are now available for a variety of both strategic and tactical
applications. Not only can they be delivered by different types of
aircraft, but rockets and guided missiles of many sizes can now carry
nuclear warheads and can be launched from the ground, the air, or
underwater. Large rockets can carry multiple warheads for delivery to
separate targets.
![[The first ICBM thermonuclear warhead]](PU/sci9icbm.gif)
The first USSR's ICBM thermonuclear warhead
Up to 3 Mt yield. Up to 8500 km flight range.
In service in 1960-1966
The Atom Bomb
The atomic bomb works by a physical phenomenon known as
fission. In this case, particles, specifically nuclei, are split and
great amounts of energy are released. This energy is expelled
explosively and violently in the atomic bomb. The massive power behind
the reaction in an atomic bomb arises from the forces that hold the atom
together called the strong nuclear force.
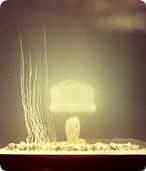
The element used in atomic bombs is Uranium-235. Uranium's atoms are
unusually large, and henceforth, it is hard for them to hold together
firmly. This makes Uranium-235 an exceptional candidate for nuclear
fission. Uranium is a heavy metal and has many more neutrons than
protons. This does not enhance their capacity to split, but it does have
an important bearing on their capacity to facilitate an explosion.
Uranium is not the only material used for making atomic bombs.
Another material is the element Plutonium, in its isotope Pu-239.
However, Plutonium will not start a fast chain reaction by itself. The
material is not fissionable in and of itself, but may act as a catalyst
to the greater reaction. The bomb basically works with a detonating head
starting off the explosive chain reaction.
The Chain Reaction
When a uranium or other suitable nucleus fissions, it breaks up into
a pair of nuclear fragments and releases energy. At the same time, the
nucleus emits very quickly a number of fast neutrons, the same type of
particle that initiated the fission of the uranium nucleus. This makes
it possible to achieve a self-sustaining series of nuclear fissions;
the neutrons that are emitted in fission produce a chain reaction,
with continuous release of energy.
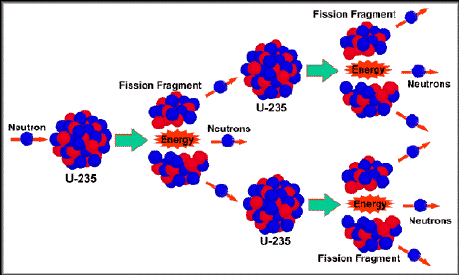
Fission of uranium 235 nucleus.
When a U-235 atom splits, it gives off energy in the form of heat and
Gamma radiation, which is the most powerful form of radioactivity and
the most lethal. When this reaction occurs, the split atom will also
give off two or three of its "spare" neutrons, which are not needed to
make either of the parts after splitting. These spare neutrons fly out
with sufficient force to split other atoms they come in contact with. In
theory, it is necessary to split only one U-235 atom, and the neutrons
from this will split other atoms, which will split more...so on and so
forth. This progression does not take place arithmetically, but
geometrically. All of this will happen within a millionth of a second.
Critical Mass
A small sphere of pure fissile material, such as uranium-235, about
the size of a golf ball, would not sustain a chain reaction. Too many
neutrons escape through the surface area, which is relatively large
compared with its volume, and thus are lost to the chain reaction. In a
mass of uranium-235 about the size of a baseball, however, the number of
neutrons lost through the surface is compensated for by the neutrons
generated in additional fissions taking place within the sphere. The
minimum amount of fissile material (of a given shape) required to
maintain the chain reaction is known as the critical mass.
Increasing the size of the sphere produces a supercritical assembly, in
which the successive generations of fissions increase very rapidly,
leading to a possible explosion as a result of the extremely rapid
release of a large amount of energy.
In an atomic bomb, therefore, a mass of fissile material greater than
the critical mass must be assembled instantaneously and held together
for about a millionth of a second to permit the chain reaction to
propagate before the bomb explodes. A heavy material, called a tamper,
surrounds the fissile mass and prevents its premature disruption. The
tamper also reduces the number of neutrons that escape.
If every atom in 0.5 kg (1.1 lb) of uranium were to split, the energy
produced would equal the explosive power of 9.9 kilotons of TNT. In this
hypothetical case, the efficiency of the process would be 100 percent.
In the first A-bomb tests, this kind of efficiency was not approached.
Moreover, a 0.5-kg (1.1-lb) mass is too small for a critical assembly.
Detonation of Atomic Bombs
Various systems have been devised to detonate the atomic bomb. The
simplest system is the gun-type weapon, in which a projectile
made of fissile material is fired at a target of the same material so
that the two weld together into a supercritical assembly. The atomic
bomb exploded by the United States over Hiroshima, Japan, on August 6,
1945, was a gun-type weapon. It had the energy of anywhere between 12.5
and 15 kilotons of TNT. Three days later the United States dropped a
second atomic bomb over Nagasaki, Japan, with the energy equivalent of
about 20 kilotons of TNT.
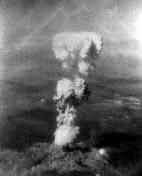 |
On August 6, 1945, an American B-29 bomber
named the Enola Gay, dropped atomic bomb on Hiroshima.
The U-235 gun-type bomb, named Little Boy, exploded at
8:16:02 a.m. In an instant 140,000 people were killed and 100,000
more were seriously injured. |
A more complex method, known as implosion, is used in a
spherically shaped weapon. The outer part of the sphere consists of a
layer of closely fitted and specially shaped devices, called lenses,
consisting of high explosive and designed to concentrate the blast
toward the center of the bomb. Each segment of the high explosive is
equipped with a detonator, which in turn is wired to all other segments.
An electrical impulse explodes all the chunks of high explosive
simultaneously, resulting in a detonation wave that converges toward the
core of the weapon. At the core is a sphere of fissile material, which
is compressed by the powerful, inwardly directed pressure, or implosion.
The density of the metal is increased, and a supercritical assembly is
produced.
The Alamogordo test bomb, as well as the one dropped by the United
States on Nagasaki, Japan, on August 9, 1945, were of the implosion type.
Each was equivalent to about 20 kilotons of TNT.
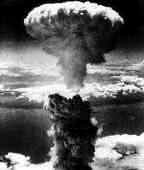 |
On August 9, 1945, the American B-29 bomber,
Bock's Car dropped Fat Man, a plutonium implosion-type bomb on
Nagasaki.
Of the 286,00 people living in Nagasaki at the time of the
blast, 74,000 were killed and another 75,000 sustained severe
injuries. |
Regardless of the method used to attain a supercritical
assembly, the chain reaction proceeds for about a millionth of a second,
liberating vast amounts of heat energy. The extremely fast release of a
very large amount of energy in a relatively small volume causes the
temperature to rise to tens of millions of degrees. The resulting rapid
expansion and vaporization of the bomb material causes a powerful
explosion.
The Hydrogen Bomb
The Hydrogen bomb works on a different physical principle known as
nuclear fusion. In nuclear fusion, the nuclei of atoms join together,
or fuse to form a heavier nucleus (specifically,
nuclei of the isotopes of hydrogen combine to form a heavier helium
nucleus). This happens only under very hot conditions. The
explosion of an atomic bomb attached to a hydrogen bomb provides the
heat to start fusion.
Fusion releases energy due to the overall loss in mass. The total of
the masses of the particles which go into a fusion reaction is bigger
than the total of the masses of the particles which come out.
The "mass difference" takes the form of energy.
The hydrogen bomb is thousands of times more powerful than an
atomic bomb. There have not been any hydrogen bombs used in warfare,
however there have been hydrogen bomb tests. Most of these tests are
done underwater due to risk of destruction.
The H-bomb is extremely powerful. A common hydrogen bomb has
the power of up to 10 megatons. This atomic bomb dropped on Hiroshima,
Japan which killed over 140,000 people had the power of 13 kilotons. All
the explosions in World War II totaled "only" 2 megatons - 20% of the
power of ONE common hydrogen bomb.
Thermonuclear Tests
On November 1, 1952, a full-scale, successful experiment was
conducted by the United States with a fusion-type device. This test,
called Mike, which was part of Operation Ivy, produced an explosion with
power equivalent to several million tons of TNT (that is, several
megatons).
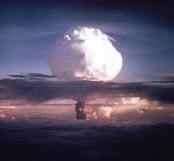 |
The first U.S. hydrogen bomb test, Mike, is
shown in the Pacific at Eniwetok Atoll, Marshall Islands, November
1, 1952.
|
![[The world's first hydrogen bomb]](PU/sci9sovhb.gif) |
The Soviet Union detonated a thermonuclear
weapon on August 12, 1953 at the Semipalatinsk test range. Up to
400 kt yield. |
On March 1, 1954, the United States exploded a fusion
bomb with a power of 15 megatons. It created a glowing fireball, more
than 4.8 km (more than 3 mi) in diameter, and a huge mushroom cloud,
which quickly rose into the stratosphere.
The March 1954 explosion led to worldwide recognition of the nature of
radioactive fallout. The fallout of radioactive debris from the huge
bomb cloud also revealed much about the nature of the thermonuclear bomb.
Had the bomb been a weapon consisting of an A-bomb trigger and a core of
hydrogen isotopes, the only persistent radioactivity from the explosion
would have been the result of the fission debris from the trigger and
from the radioactivity induced by neutrons in coral and seawater. Some
of the radioactive debris, however, fell on the Lucky Dragon, a Japanese
vessel engaged in tuna fishing about 160 km (about 100 mi) from the test
site. This radioactive dust was later analyzed by Japanese scientists.
The results demonstrated that the bomb that dusted the Lucky Dragon with
fallout was more than just an H-bomb.
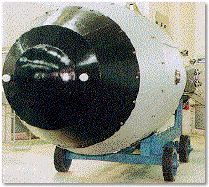 |
The world's most powerful
experimental bomb.
Tested on November 30, 1961 at the Novaya Zemlya test range.
More that 100 Mt rated yield.
Test-detonated at half the yield. |
Fission-Fusion-Fission Bomb
The thermonuclear bomb exploded in 1954 was a three-stage
weapon. The first stage consisted of a big A-bomb, which acted as a
trigger. The second stage was the H-bomb phase resulting from the fusion
of deuterium and tritium within the bomb. In the process helium and high-energy
neutrons were formed. The third stage resulted from the impact of these
high-speed neutrons on the outer jacket of the bomb, which consisted of
natural uranium, or uranium-238. No chain reaction was produced, but the
fusion neutrons had sufficient energy to cause fission of the uranium
nuclei and thus added to the explosive yield and also to the
radioactivity of the bomb residues.
The Neutron Bomb - Clean H-Bomb
On the average, about 50 percent of the power of an H-bomb
results from thermonuclear-fusion reactions and the other 50 percent
from fission that occurs in the A-bomb trigger and in the uranium jacket.
A clean H-bomb is defined as one in which a significantly smaller
proportion than 50 percent of the energy arises from fission. Because
fusion does not produce any radioactive products directly, the fallout
from a clean weapon is less than that from a normal or average H-bomb of
the same total power. If an H-bomb were made with no uranium jacket but
with a fission trigger, it would be relatively clean. Perhaps as little
as 5 percent of the total explosive force might result from fission; the
weapon would thus be 95 percent clean. The enhanced-radiation fusion
bomb, also called the neutron bomb, which has been tested by the United
States and other nuclear powers, does not release long-lasting
radioactive fission products. However, the large number of neutrons
released in thermonuclear reactions is known to induce radioactivity in
materials, especially earth and water, within a relatively small area
around the explosion.
Thus the neutron bomb is considered a tactical weapon because it can do
serious damage on the battlefield, penetrating tanks and other armored
vehicles and causing death or serious injury to exposed individuals,
without producing the radioactive fallout that endangers people or
structures miles away.
Effects of Nuclear Weapons
Nuclear Explosions produce both immediate and delayed destructive
effects. Blast, thermal radiation, prompt ionizing radiation are
produced and cause significant destruction within seconds or minutes of
a nuclear detonation. The delayed effects, such as radioactive fallout
and other possible environmental effects, inflict damage over an
extended period ranging from hours to years.
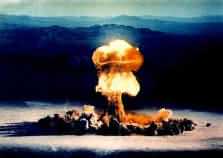
Destruction of a military target
by a nuclear fission weapon is comprehended mainly by the effects
of a powerful blast wave in air but the more distant military
consequences of a nuclear explosion can be significantly augmented
by the thermal (heat) radiations emitted by the fireball that
emerges from the isothermal sphere. Furthermore, accurate
anticipation of the thermal characteristics and physical behavior
of an isothermal sphere as it endures and rises above a nuclear
explosion can be useful as a basis to predict the military and
environmental effects of radioactive fallout since most of the
dangerous fission byproducts of an atomic bomb detonation are
concentrated in the isothermal sphere. |
The effects of nuclear weapons were carefully observed,
both after the bombings of Hiroshima and Nagasaki and after many test
explosions in the 1950s and early 1960s. The basic effects of nuclear
explosion are:
- Blast Effects
- Thermal Effects
- Radiation
- Electromagnetic pulse (EMP)
- Fallout
- Climatic Effects
As is the case with explosions caused by conventional
weapons, most of the damage to buildings and other structures from a
nuclear explosion results, directly or indirectly, from the effects of
blast.
The very rapid expansion of the bomb materials produces
a high-pressure pulse, or shock wave, that moves rapidly outward from
the exploding bomb. In air, this shock wave is called a blast wave
because it is equivalent to and is accompanied by powerful winds of much
greater than hurricane force. Damage is caused both by the high excess (or
overpressure) of air at the front of the blast wave and by the extremely
strong winds that persist after the wave front has passed.
In general, large buildings are destroyed by the change in air pressure,
while people and objects such as trees and utility poles are destroyed
by the wind.
The degree of blast damage suffered on the ground
depends on:
-
TNT equivalent of the explosion;
-
the altitude at which the bomb is exploded (the height
of burst);
-
and the distance of the structure from ground zero (the
point directly under the bomb).
Assuming a height of burst that will maximize the damage
area, a 10-kiloton bomb will cause severe damage to wood-frame houses,
such as are common in the United States, to a distance of more than 1.6
km (more than 1 mi) from ground zero and moderate damage as far as 2.4
km (1.5 mi). (A severely damaged house probably would be beyond repair.)
The damage radius increases with the power of the bomb, approximately in
proportion to its cube root. If exploded at the optimum height,
therefore, a 10-megaton weapon, which is 1,000 times as powerful as a
10-kiloton weapon, will increase the distance tenfold, that is, out to
17.7 km (11 mi) for severe damage and 24 km (15 mi) for moderate damage
of a frame house.
When a nuclear weapon is detonated on or near Earth's
surface, the blast digs out a large crater. Some of the material that
used in be in the crater is deposited on the rim of the crater; the rest
is carried up into the air and returns to Earth as radioactive fallout.
An explosion that is farther above the Earth's surface than the radius
of the fireball does not dig a crater and produces negligible immediate
fallout. For the most part, a nuclear blast kills people by indirect
means rather than by direct pressure.
Approximately 35 percent of the energy from a nuclear
explosion is an intense burst of thermal radiation, i.e., heat. The
effects are similar to the effect of a two-second flash from an enormous
sunlamp. The very high temperatures attained in a nuclear explosion
result in the formation of an extremely hot incandescent mass of gas
called a fireball.
For a 10-kiloton explosion in the air, the fireball will attain a
maximum diameter of about 300 m (about 1,000 ft); for a 10-megaton
weapon the fireball may be 4.8 km (3 mi) across. A flash of thermal (or
heat) radiation is emitted from the fireball and spreads out over a
large area, but with steadily decreasing intensity. The amount of heat
energy received a certain distance from the nuclear explosion depends on
the power of the weapon and the state of the atmosphere. If the
visibility is poor or the explosion takes place above clouds, the
effectiveness of the heat flash is decreased.
The thermal radiation falling on exposed skin can cause what are called
flash burns. A 10-kiloton explosion in the air can produce
moderate (second-degree) flash burns, which require some medical
attention, as far as 2.4 km (1.5 mi) from ground zero; for a 10-megaton
bomb, the corresponding distance would be more than 32 km (more than 20
mi). Milder burns of bare skin would be experienced even farther out.
Most ordinary clothing provides protection from the heat radiation, as
does almost any opaque object. Flash burns occur only when the bare skin
is directly exposed, or if the clothing is too thin to absorb the
thermal radiation.
The heat radiation can initiate fires in dry, flammable materials, for
example, paper and some fabrics, and such fires may spread if conditions
are suitable.
The evidence from the A-bomb explosions over Japan indicates that many
fires, especially in the area near ground zero, originated from
secondary causes, such as electrical short circuits, broken gas lines,
and upset furnaces and boilers in industrial plants. The blast damage
produced debris that helped to maintain the fires and denied access to
fire-fighting equipment. Thus, much of the fire damage in Japan was a
secondary effect of the blast wave.
Under some conditions, such as existed at Hiroshima but not at Nagasaki,
many individual fires can combine to produce a fire storm similar to
those that accompany some large forest fires. The heat of the fire
causes a strong updraft, which produces strong winds drawn in toward the
center of the burning area. These winds fan the flame and convert the
area into a holocaust in which everything flammable is destroyed.
Inasmuch as the flames are drawn inward, however, the area over which
such a fire spreads may be limited.
Since the thermal radiation travels at roughly the speed
of light, the flash of light and heat precedes the blast wave by several
seconds, just as lightning is seen before thunder is heard.
The visible light will produce "flashblindness" in people who are
looking in the direction of the explosion. Flashblindness can last for
several minutes, after which recovery is total. If the flash is focused
through the lens of the eye, a permanent retinal burn will result. At
Hiroshima and Nagasaki, there were many cases of flashblindness, but
only one case of retinal burn, among the survivors. On the other hand,
anyone flashblinded while driving a car could easiIy cause permanent
injury to himself and to others.
Besides heat and blast, an exploding nuclear bomb has a
unique effect—it releases penetrating nuclear radiation, which is quite
different from thermal (or heat) radiation. Direct radiation occurs at
the time of the explosion. It can be very intense, but its range is
limited. For large nuclear weapons, the range of intense direct
radiation is less than the range of lethal blast and thermal radiation
effects. However, in the case of smaller weapons, direct radiation may
be the lethal effect with the greatest range. Direct radiation did
substantial damage to the residents of Hiroshima and Nagasaki. Human
response to ionizing radiation is subject to great scientific
uncertainty and intense controversy. It seems likely that even small
doses of radiation do some harm.
When absorbed by the body, nuclear radiation can cause serious injury.
For an explosion high in the air, the injury range for these radiations
is less than for blast and fire damage or flash burns. In Japan, however,
many individuals who were protected from blast and burns succumbed later
to radiation injury.
Nuclear radiation from an explosion may be divided into two categories,
namely, prompt radiation and residual radiation. The prompt
radiation consists of an instantaneous burst of neutrons and gamma rays,
which travel over an area of several square miles. Gamma rays are
identical in effect to X rays (see X Ray). Both neutrons and gamma rays
have the ability to penetrate solid matter, so that substantial
thicknesse of shielding materials are required.
The residual nuclear radiation, generally known as fallout, can be a
hazard over very large areas that are completely free from other effects
of a nuclear explosion. In bombs that gain their energy from fission of
uranium-235 or plutonium-239, two radioactive nuclei are produced for
every fissile nucleus split. These fission products account for the
persistent radioactivity in bomb debris, because many of the atoms have
half-lives measured in days, months, or years.
Two distinct categories of fallout, namely, early and delayed, are known.
If a nuclear explosion occurs near the surface, earth or water is taken
up into a mushroom-shaped cloud and becomes contaminated with the
radioactive weapon residues. The contaminated material begins to descend
within a few minutes and may continue to fall for about 24 hours,
covering an area of thousands of square miles downwind from the
explosion. This constitutes the early fallout, which is an immediate
hazard to human beings. No early fallout is associated with high-altitude
explosions. If a nuclear bomb is exploded well above the ground, the
radioactive residues rise to a great height in the mushroom cloud and
descend gradually over a large area.
Human experience with radioactive fallout has been minimal. The
principal known case histories have been derived from the accidental
exposure of fishermen and local residents to the fallout from the
15-megaton explosion that occurred on March 1, 1954. The nature of
radioactivity, however, and the immense areas contaminable by a single
bomb undoubtedly make radioactive fallout potentially one of the most
lethal effects of nuclear weapons.
Spectacular photo album of
Nuclear Explosions
Electromagnetic pulse (EMP) is an electromagnetic wave
similar to radio waves, which results from secondary reactions occurring
when the nuclear gamma radiation is absorbed in the air or ground. It
differs from the usual radio waves in two important ways. First, it
creates much higher electric field strengths. Whereas a radio signal
might produce a thousandth of a volt or less in a receiving antenna, an
EMP pulse might produce thousands of volts. Secondly, it is a single
pulse of energy that disappears completely in a small fraction of a
second. In this sense, it is rather similar to the electrical signal
from lightning, but the rise in voltage is typically a hundred times
faster. This means that most equipment designed to protect electrical
facilities from lightning works too slowly to be effective against EMP.
An attacker might detonate a few weapons at high
altitudes in an effort to destroy or damage the communications and
electric power systems of the victim. There is no evidence that EMP is a
physical threat to humans. However, electrical or electronic systems,
particularly those connected to long wires such as power lines or
antennas, can undergo damage. There could be actual physical damage
to an electrical component or a temporary disruption of operation.
Fallout radiation is received from particles that are
made radioactive by the effects of the explosion, and subsequently
distributed at varying distances from the site of the blast. While any
nuclear explosion in the atmosphere produces some fallout, the fallout
is far greater if the burst is on the surface, or at least low enough
for the firebal to touch the ground. The significant hazards come from
particles scooped up from the ground and irradiated by the nuclear
explosion. The radioactive particles that rise only a short distance (those
in the "stem" of the familiar mushroom cloud) will fall back to earth
within a matter of minutes, landing close to the center of the explosion.
Such particles are unlikely to cause many deaths, because they will fall
in areas where most people have already been killed. However, the
radioactivity will complicate efforts at rescue or eventual
reconstruction. The radioactive particles that rise higher will be
carried some distance by the wind before returning to Earth, and hence
the area and intensity of the fallout is strongly influenced by local
weather conditions. Much of the material is simply blown downwind in a
long plume. Rainfall also can have a significant influence on the ways
in which radiation from smaller weapons is deposited, since rain will
carry contaminated particles to the ground. The areas receiving such
contaminated rainfall would become "hot spots," with greater radiation
intensity than their surroundings.
Besides the blast and radiation damage from individual
bombs, a large-scale nuclear exchange between nations could conceivably
have a catastrophic global effect on climate. This possibility, proposed
in a paper published by an international group of scientists in December
1983, has come to be known as the "nuclear winter" theory. According to
these scientists, the explosion of not even one-half of the combined
number of warheads in the United States and Russia would throw enormous
quantities of dust and smoke into the atmosphere. The amount could be
sufficient to block off sunlight for several months, particularly in the
northern hemisphere, destroying plant life and creating a subfreezing
climate until the dust dispersed. The ozone layer might also be affected,
permitting further damage as a result of the sun's ultraviolet radiation.
Were the results sufficiently prolonged, they could spell the virtual
end of human civilization. The nuclear winter theory has since become
the subject of enormous controversy. It found support in a study
released in December 1984 by the U.S. National Research Council, and
other groups have undertaken similar research. In 1985, however, the
U.S. Department of Defense released a report acknowledging the validity
of the concept but saying that it would not affect defense policies.
Alıntı:
http://www.world-mysteries.com/sci_9.htm
Hiçbir
yazı/ resim izinsiz olarak kullanılamaz!! Telif hakları uyarınca
bu bir suçtur..! Tüm hakları Çetin BAL' a aittir. Kaynak gösterilmek şartıyla siteden
alıntı yapılabilir.
The Time Machine Project © 2005 Cetin BAL - GSM:+90 05366063183 -Turkiye/Denizli
Ana Sayfa /İndex /Roket bilimi /
E-Mail /CetinBAL /Quantum Teleportation-2
Time Travel Technology /Ziyaretçi
Defteri /
Duyuru
/ UFO Technology
Kuantum Teleportation /
Kuantum Fiziği
/Astronomy
|